Warming and sea ice loss over the past few decades have caused major changes in sea surface partial pressure of CO2 (pCO2) of the western Arctic Ocean, but detailed temporal variations and trends during this period of rapid climate-driven changes are not well known.
Based on an analysis of an international Arctic pCO2 synthesis data set collected between 1994-2017, the authors of a recent paper published in Nature Climate Change observed that summer sea surface pCO2 in the Canada Basin is increasing at twice the rate of atmospheric CO2 rise. Warming, ice loss and subsequent CO2 uptake in the Basin are amplifying seasonal pCO2 changes, resulting in a rapid long-term increase. Consequently, the summer air-sea CO2 gradient has decreased sharply and may approach zero by the 2030s, which is reducing the basin’s capacity to remove CO2 from the atmosphere. In stark contrast, sea surface pCO2 on the Chukchi Shelf remains low and relatively constant during this time frame, which the authors attribute to increasingly strong biological production in response to higher intrusion of nutrient-rich Pacific Ocean water onto the shelf as a result of increased Bering Strait throughflow. These trends suggest that, unlike the Canada Basin, the Chukchi Shelf will become a larger carbon sink in the future, with implications for the deep ocean carbon cycle and ecosystem.
As Arctic sea ice melting accelerates, more fresh, low-buffer capacity, high-CO2 water will enter the upper layer of the Canada Basin, which may rapidly acidify the surface water, endanger marine calcifying organisms, and disrupt ecosystem function.
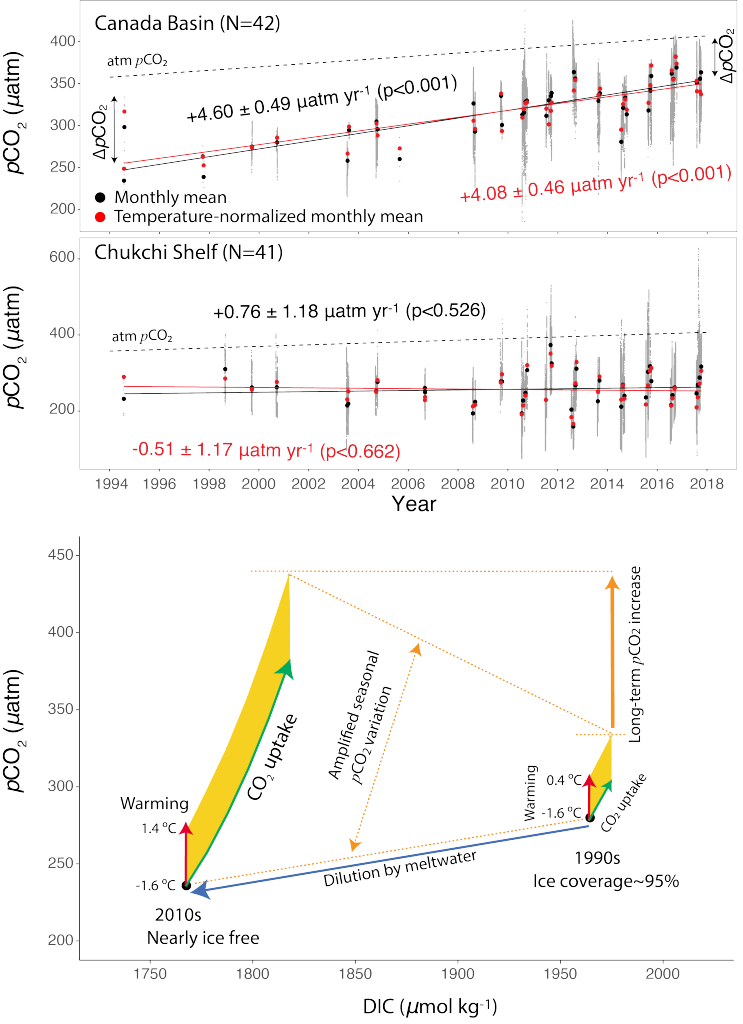
Figure. 1: TOP) Sea surface pCO2 trend in the Canada Basin and Chukchi Shelf. The grey dots represent the raw observations of pCO2, black dots are the monthly mean of pCO2 at in situ SST, and red dots are the monthly means of pCO2 normalized to the long-term means of SST. The arrows indicate the statistically significant change in ∆pCO2. BOTTOM) Sea ice-loss amplifying surface water pCO2 in the Canada Basin. Black dots represent the initial condition for pCO2 and DIC at -1.6 ℃. The arrows indicate the processes of warming (red), CO2 uptake from the atmosphere (green), dilution by ice meltwater (blue). The yellow shaded areas indicate the possible seasonal variations of pCO2, which are amplified by the synergistic effect of ice melt, warming and CO2 uptake.
Authors:
Zhangxian Ouyang (University of Delaware, USA),
Di Qi (Third Institute of Oceanography, China),
Liqi Chen (Third Institute of Oceanography, China),
Taro Takahashi† (Columbia University, USA),
Wenli Zhong (Ocean University of China, China),
Michael D. DeGrandpre (University of Montana, USA),
Baoshan Chen (University of Delaware, USA),
Zhongyong Gao (Third Institute of Oceanography, China),
Shigeto Nishino (Japan Agency for Marine-Earth Science and Technology, Japan),
Akihiko Murata (Japan Agency for Marine-Earth Science and Technology, Japan),
Heng Sun (Third Institute of Oceanography, China),
Lisa L. Robbins (University of South Florida, USA),
Meibing Jin (International Arctic Research Center, USA),
Wei-Jun Cai* (University of Delaware, USA)