Phytoplankton provide the energy that fuels marine food webs, yet differences in fisheries catch across global ecosystems far exceed accompanying differences in phytoplankton production. Nearly 50 years ago, John Ryther hypothesized that this contrast must arise from synergistic interactions between phytoplankton production and food webs. New perspectives on global fish catch, fishing effort, and a prototype high-resolution global earth system model allowed us to revisit Ryther’s supposition and explore its implications under climate change. After accounting for a small number of lightly fished ecosystems, we find that stark differences in regional catch can be explained with an energetically constrained model that a) resolves large inter-regional differences in the benthic and pelagic energy pathways connecting phytoplankton and fish; b) reduces trophic transfer efficiencies in warm, tropical ecosystems; and, less critically, c) associates elevated trophic transfer efficiencies with benthic systems. The same food web processes that accentuate spatial differences in phytoplankton production in the contemporary ocean also accentuated temporal trends under climate change, with projected fish catch changes in some areas exceeding 50% (Figure 1). Our results, recently published in PNAS, demonstrate the importance of marine resource management strategies that are robust to potentially significant changes in fisheries productivity baselines. These results also provide impetus for efforts to improve constraints on regional ocean productivity projections that often disagree in present earth system models.
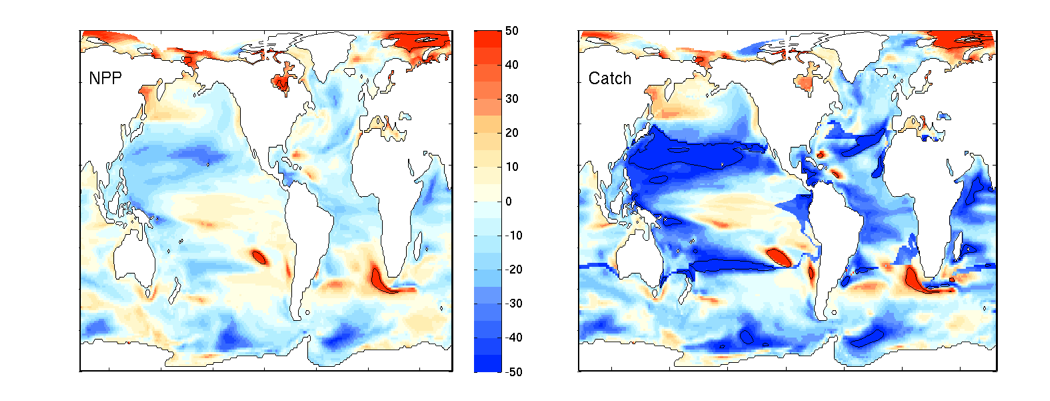
Figure 1: Projected percent changes in net phytoplankton production (left) and fisheries catch (right) between 2050-2100 and 1950-2000 under a high greenhouse gas emission scenario (RCP8.5) in GFDL’s ESM2M-COBALT Earth System Model. Contours are shown for +/- 50%.
Authors: Charles A. Stocka, Jasmin G. Johna, Ryan R. Rykaczewskib,c, Rebecca G. Aschd, William W.L. Cheunge, John P. Dunnea, Kevin D. Friedlandf, Vicky W.Y. Lame, Jorge L. Sarmientod, and Reg A. Watsong
aGeophysical Fluid Dynamics Laboratory, National Oceanic and Atmospheric Administration
bSchool of the Earth, Ocean, and Environment, University of South Carolina
cDepartment of Biological Sciences, University of South Carolina
dAtmospheric and Oceanic Sciences Program, Princeton University
eNippon Foundation-Nereus Program, Institute of Oceans and Fisheries, The University of British Columbia
fNational Marine Fisheries Service, Narragansett, RI
gInstitute for Marine and Antarctic Studies, University of Tasmania, Australia