Phytoplankton respond directly to climate forcing, and due to their central role in global oxygen production and atmospheric carbon sequestration, they are critical components of the Earth’s climate system. There are however few observations detailing past variability in marine primary productivity, particularly over multi-decadal to centennial timescales. This limits our understanding of the long-term impact of climatic forcing on both past and future marine productivity.
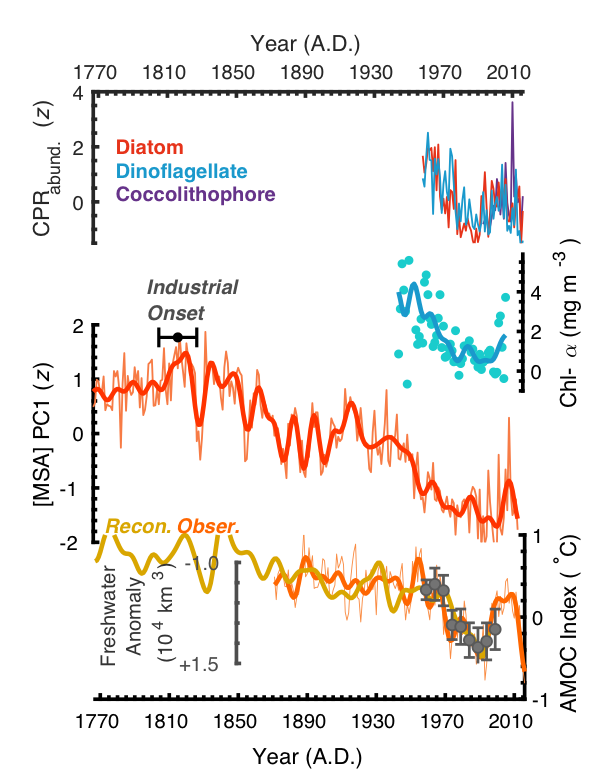
Multi-century decline of subarctic Atlantic productivity. From top: standardized (z-score units relative to ad 1958-2016) indices of Continuous Plankton Recorder (CPR)-based diatom, dinoflagellate and coccolithophore relative-abundances; North Atlantic [chlorophyll-α] reconstruction from Boyce et al. (2010, Nature); ice core-based [MSA] PC1 productivity index. The “Industrial Onset” range shows the estimated initiation of declining subarctic Atlantic productivity; reconstructed (Rahmstorf et al., 2015, Nat. Clim. Change) and observed sea-surface temperature-based Atlantic Meridional Overturning Circulation (i.e., AMOC) index, alongside 5-year averaged subarctic Atlantic freshwater storage anomalies (relative to A.D. 1955) from Curry and Mauritzen (2005; Science).
Future atmospheric warming is predicted to contribute to accelerating Greenland Ice Sheet runoff, ocean-surface freshening, and AMOC slowdown, suggesting the potential for continued declines in productivity across this dynamic and climatically important region. Such declines will, in turn, have important implications for future maritime economies, global food security, and drawdown of atmospheric carbon dioxide.
Authors:
Matthew Osman (Massachusetts Institute of Technology)
Sarah Das (Woods Hole Oceanographic Institution)
Luke Trusel (Rowan University)
Matthew Evans (Wheaton College)
Hubertus Fischer (University of Bern)
Mackenzie Griemann (University of California, Irvine)
Sepp Kipfstuhl (Alfred-Wegener-Institute)
Joseph McConnell (Desert Research Institute)
Eric Saltzman (University of California, Irvine)
Figure references:
Boyce, D. G., Lewis, M. R. & Worm, B. (2010) Global phytoplankton decline over the past century. Nature 466, 591–596.
Curry, R. & Mauritzen, C. (2005) Dilution of the northern North Atlantic Ocean in recent decades. Science 308, 1772–1774.
Rahmstorf, S. et al. (2015) Exceptional twentieth-century slowdown in Atlantic Ocean overturning circulation. Nat. Clim. Change 5, 475–480.